Roles of RNA silencing in viral and non-viral plant immunity and in the crosstalk between disease resistance systems
RNA silencing is a well-established antiviral immunity system in plants, in which small RNAs guide Argonaute proteins to targets in viral RNA or DNA, resulting in virus repression. Virus-encoded suppressors of silencing counteract this defence system. In this Review, we discuss recent findings about antiviral RNA silencing, including the movement of RNA through plasmodesmata and the differentiation between plant self and viral RNAs. We also discuss the emerging role of RNA silencing in plant immunity against non-viral pathogens. This immunity is mediated by transkingdom movement of RNA into and out of the infected plant cells in vesicles or as extracellular nucleoproteins and, like antiviral immunity, is influenced by the silencing suppressors encoded in the pathogens’ genomes. Another effect of RNA silencing on general immunity involves host-encoded small RNAs, including microRNAs, that regulate NOD-like receptors and defence signalling pathways in the innate immunity system of plants. These RNA silencing pathways form a network of processes with both positive and negative effects on the immune systems of plants.
This is a preview of subscription content, access via your institution
Access options
Access Nature and 54 other Nature Portfolio journals
Get Nature+, our best-value online-access subscription
cancel any time
Subscribe to this journal
Receive 12 print issues and online access
206,07 € per year
only 17,17 € per issue
Buy this article
- Purchase on SpringerLink
- Instant access to full article PDF
Prices may be subject to local taxes which are calculated during checkout
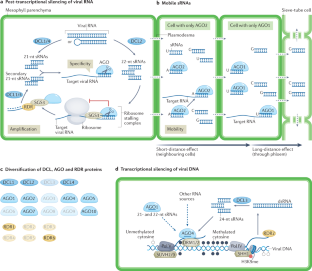
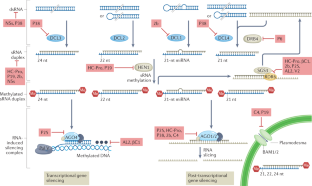
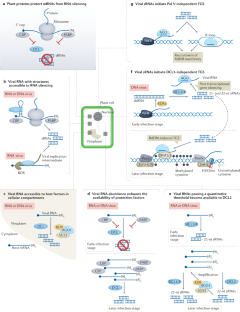

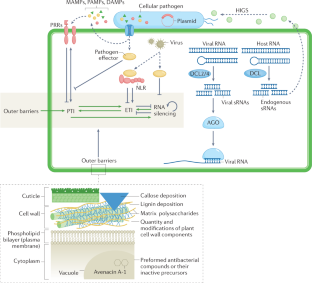
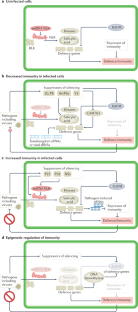
Similar content being viewed by others
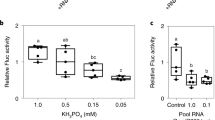
Exogenous miRNAs induce post-transcriptional gene silencing in plants
Article Open access 14 October 2021
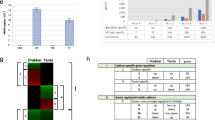
Turnip mosaic virus in oilseed rape activates networks of sRNA-mediated interactions between viral and host genomes
Article Open access 23 November 2020
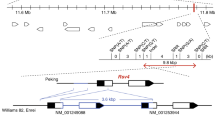
Soybean antiviral immunity conferred by dsRNase targets the viral replication complex
Article Open access 27 September 2019
References
- Baulcombe, D. RNA silencing in plants. Nature431, 356–363 (2004). ArticleCASPubMedGoogle Scholar
- Ding, S. W. RNA-based antiviral immunity. Nat. Rev. Immunol.10, 632–644 (2010). ArticleCASPubMedGoogle Scholar
- Csorba, T., Kontra, L. & Burgyán, J. Viral silencing suppressors: tools forged to fine-tune host-pathogen coexistence. Virology479–480, 85–103 (2015). ArticlePubMedCASGoogle Scholar
- Valli, A. A., Gallo, A., Rodamilans, B., López-Moya, J. J. & García, J. A. The HCPro from the Potyviridae family: an enviable multitasking helper component that every virus would like to have. Mol. Plant. Pathol.19, 744–763 (2018). ArticlePubMedGoogle Scholar
- Gaffar, F. Y. & Koch, A. Catch me if you can! RNA silencing-based improvement of antiviral plant immunity. Viruses11, 673 (2019). ArticleCASPubMed CentralGoogle Scholar
- Maillard, P. V., Veen, A. G., van der, Poirier, E. Z. & e.Sousa, C. R. Slicing and dicing viruses: antiviral RNA interference in mammals. EMBO J.38, e100941 (2019). ArticleCASPubMedPubMed CentralGoogle Scholar
- Bonning, B. C. & Saleh, M. C. The interplay between viruses and RNAi pathways in insects. Annu. Rev. Entomol.66, 61–79 (2021). ArticleCASPubMedGoogle Scholar
- Lee, C. H. & Carroll, B. J. Evolution and diversification of small RNA pathways in flowering plants. Plant. Cell Physiol.59, 2169–2187 (2018). CASPubMedGoogle Scholar
- Borges, F. & Martienssen, R. A. The expanding world of small RNAs in plants. Nat. Rev. Mol. Cell Biol.16, 727–741 (2015). ArticleCASPubMedPubMed CentralGoogle Scholar
- Anderson, J. P. et al. Plants versus pathogens: an evolutionary arms race. Funct. plant. Biol.37, 499 (2010). ArticlePubMedPubMed CentralGoogle Scholar
- Cui, J., You, C. & Chen, X. The evolution of microRNAs in plants. Curr. Opin. Plant. Biol.35, 61–67 (2017). ArticleCASPubMedGoogle Scholar
- Margis, R. et al. The evolution and diversification of Dicers in plants. Febs Lett.580, 2442–2450 (2006). ArticleCASPubMedGoogle Scholar
- Wu, H. et al. Plant 22-nt siRNAs mediate translational repression and stress adaptation. Nature581, 89–93 (2020). This study shows that 22-nucleotide sRNAs trigger amplification of silencing, and provides evidence for an effect on translation regulation. ArticleCASPubMedGoogle Scholar
- Cuperus, J. T. et al. Unique functionality of 22-nt miRNAs in triggering RDR6-dependent siRNA biogenesis from target transcripts in Arabidopsis. Nat. Struct. Mol. Biol.17, 997–1003 (2010). ArticleCASPubMedPubMed CentralGoogle Scholar
- Chen, H.-M. et al. 22-nucleotide RNAs trigger secondary siRNA biogenesis in plants. Proc. Natl Acad. Sci. USA107, 15269–15274 (2010). ArticleCASPubMedPubMed CentralGoogle Scholar
- MacLean, D. et al. Evidence for large complex networks of plant short silencing RNAs. PLoS ONE5, e9901 (2010). ArticlePubMedPubMed CentralCASGoogle Scholar
- Iwakawa, H. O. et al. Ribosome stalling caused by the Argonaute-microRNA-SGS3 complex regulates the production of secondary siRNAs in plants. Cell Rep.35, 109300 (2021). ArticleCASPubMedGoogle Scholar
- Wang, Z. et al. A novel DCL2-dependent miRNA pathway in tomato affects susceptibility to RNA viruses. Genes Dev.32, 1155–1160 (2018). ArticleCASPubMedPubMed CentralGoogle Scholar
- Wang, T. et al. Tomato DCL2b is required for the biosynthesis of 22-nt small RNAs, the resulting secondary siRNAs, and the host defense against ToMV. Hortic. Res.5, 62 (2018). ArticleCASPubMedPubMed CentralGoogle Scholar
- Parent, J. S., Bouteiller, N., Elmayan, T. & Vaucheret, H. Respective contributions of Arabidopsis DCL2 and DCL4 to RNA silencing. Plant. J.81, 223–232 (2015). ArticleCASPubMedGoogle Scholar
- Bouché, N., Lauressergues, D., Gasciolli, V. & Vaucheret, H. An antagonistic function for Arabidopsis DCL2 in development and a new function for DCL4 in generating viral siRNAs. EMBO J.25, 3347–3356 (2006). ArticlePubMedPubMed CentralCASGoogle Scholar
- Blevins, T. et al. Four plant Dicers mediate viral small RNA biogenesis and DNA virus induced silencing. Nucleic Acids Res.34, 6233–6246 (2006). ArticleCASPubMedPubMed CentralGoogle Scholar
- Carbonell, A. & Carrington, J. C. Antiviral roles of plant ARGONAUTES. Curr. Opin. Plant. Biol.27, 111–117 (2015). ArticleCASPubMedPubMed CentralGoogle Scholar
- Willmann, M. R. et al. The functions of RNA-dependent RNA polymerases in Arabidopsis. Arabidopsis Book.9, e0146 (2011). ArticlePubMedPubMed CentralGoogle Scholar
- Coursey, T., Regedanz, E. & Bisaro, D. M. Arabidopsis RNA polymerase V mediates enhanced compaction and silencing of geminivirus and transposon chromatin during host recovery from infection. J. Virol.92, 1–18 (2018). ArticleGoogle Scholar
- Marí-Ordóñez, A. et al. Reconstructing de novo silencing of an active plant retrotransposon. Nat. Genet.45, 1029–1039 (2013). ArticlePubMedCASGoogle Scholar
- Huang, L. F. et al. An atypical RNA polymerase involved in RNA silencing shares small subunits with RNA polymerase II. Nat. Struct. Mol. Biol.16, 91–93 (2009). ArticleCASPubMedGoogle Scholar
- Ream, T. S. et al. Subunit compositions of the RNA-silencing enzymes Pol IV and Pol V reveal their origins as specialized forms of RNA polymerase II. Mol. Cell33, 192–203 (2009). ArticleCASPubMedGoogle Scholar
- Ye, R. et al. A Dicer-independent route for biogenesis of siRNAs that direct DNA methylation in Arabidopsis. Mol. Cell61, 222–235 (2016). ArticleCASPubMedGoogle Scholar
- Yang, D.-L. et al. Dicer-independent RNA-directed DNA methylation in Arabidopsis. Cell Res.26, 66–82 (2015). ArticlePubMedPubMed CentralCASGoogle Scholar
- Matzke, M. A. & Mosher, R. A. RNA-directed DNA methylation: an epigenetic pathway of increasing complexity. Nat. Rev. Genet.15, 394–408 (2014). ArticleCASPubMedGoogle Scholar
- Johnson, L. M. et al. SRA- and SET-domain-containing proteins link RNA polymerase V occupancy to DNA methylation. Nature507, 124–128 (2014). ArticleCASPubMedPubMed CentralGoogle Scholar
- Liu, Z. W. et al. The SET domain proteins SUVH2 and SUVH9 are required for Pol V occupancy at RNA-directed DNA methylation loci. PLoS Genet.10, e1003948 (2014). ArticlePubMedPubMed CentralCASGoogle Scholar
- Johnson, L. M., Law, J. A., Khattar, A., Henderson, I. R. & Jacobsen, S. E. SRA-domain proteins required for DRM2-mediated de novo DNA methylation. PLoS Genet.4, e1000280 (2008). ArticlePubMedPubMed CentralCASGoogle Scholar
- Ding, S.-W. & Voinnet, O. Antiviral immunity directed by small RNAs. Cell130, 413–426 (2007). ArticleCASPubMedPubMed CentralGoogle Scholar
- Wang, L. et al. A virus-encoded protein suppresses methylation of the viral genome through its interaction with ago4 in the cajal body. eLife9, e55542 (2020). Previous evidence implicated RdDM in virus defence and Cajal bodies in RdDM. This article links the two through an interaction of a VSR (V2 of tomato yellow leaf curl virus) with AGO4 in the Cajal body. ArticleCASPubMedPubMed CentralGoogle Scholar
- Raja, P., Sanville, B. C., Buchmann, R. C. & Bisaro, D. M. Viral genome methylation as an epigenetic defense against geminiviruses. J. Virol.82, 8997–9007 (2008). ArticleCASPubMedPubMed CentralGoogle Scholar
- Rosas-Diaz, T. et al. A virus-targeted plant receptor-like kinase promotes cell-to-cell spread of RNAi. Proc. Natl Acad. Sci. USA115, 1388–1393 (2018). ArticleCASPubMedPubMed CentralGoogle Scholar
- Garnelo Gόmez, B. et al. The viral silencing suppressor P19 interacts with the receptor-like kinases BAM1 and BAM2 and suppresses the cell-to-cell movement of RNA silencing independently of its ability to bind sRNA. N. Phytol.229, 1840–1843 (2021). ArticleCASGoogle Scholar
- Anandalakshmi, R. et al. A calmodulin-related protein that suppresses posttranscriptional gene silencing in plants. Science290, 142–144 (2000). ArticleCASPubMedGoogle Scholar
- Li, F., Huang, C., Li, Z. & Zhou, X. Suppression of RNA silencing by a plant DNA virus satellite requires a host calmodulin-like protein to repress RDR6 expression. PLoS Pathog.10, 11–14 (2014). ArticleCASGoogle Scholar
- Yong Chung, H., Lacatus, G. & Sunter, G. Geminivirus AL2 protein induces expression of, and interacts with, a calmodulin-like gene, an endogenous regulator of gene silencing. Virology460–461, 108–118 (2014). ArticlePubMedCASGoogle Scholar
- Shamandi, N. et al. Plants encode a general siRNA suppressor that is induced and suppressed by viruses. PLoS Biol.13, e1002326 (2015). ArticlePubMedPubMed CentralCASGoogle Scholar
- Liu, L. & Chen, X. RNA quality control as a key to suppressing RNA silencing of endogenous genes in plants. Mol. Plant.9, 826–836 (2016). ArticleCASPubMedGoogle Scholar
- Heinlein, M. Plant virus replication and movement. Virology479–480, 657–671 (2015). ArticlePubMedCASGoogle Scholar
- Bologna, N. G. et al. Nucleo-cytosolic shuttling of ARGONAUTE1 prompts a revised model of the plant microRNA pathway. Mol. Cell69, 709–719 (2018). ArticleCASPubMedGoogle Scholar
- Ye, R. et al. Cytoplasmic assembly and selective nuclear import of Arabidopsis ARGONAUTE4/siRNA complexes. Mol. Cell46, 859–870 (2012). ArticleCASPubMedGoogle Scholar
- Fang, Y. & Spector, D. L. Identification of nuclear dicing bodies containing proteins for microRNA biogenesis in living Arabidopsis plants. Curr. Biol.17, 818–823 (2007). ArticleCASPubMedPubMed CentralGoogle Scholar
- Brosseau, C. & Moffett, P. Functional and genetic analysis identify a role for Arabidopsis ARGONAUTE5 in antiviral RNA silencing. Plant. Cell27, 1742–1754 (2015). ArticleCASPubMedPubMed CentralGoogle Scholar
- Zheng, X., Fahlgren, N., Abbasi, A., Berry, J. C. & Carrington, J. C. Antiviral ARGONAUTEs against turnip crinkle virus revealed by image-based trait analysis. Plant. Physiol.180, 1418–1435 (2019). ArticleCASPubMedPubMed CentralGoogle Scholar
- Lindbo, J. A., Silva-Rosales, L., Proebsting, W. M. & Dougherty, W. G. Induction of a highly specific antiviral state in transgenic plants: implications for regulation of gene expression and virus resistance. Plant. Cell5, 1749–1759 (1993). ArticleCASPubMedPubMed CentralGoogle Scholar
- Bond, D. M. & Baulcombe, D. C. Epigenetic transitions leading to heritable, RNA-mediated de novo silencing in Arabidopsis thaliana. Proc. Natl Acad. Sci. USA112, 917–922 (2015). ArticleCASPubMedPubMed CentralGoogle Scholar
- Sigman, M. J. et al. An siRNA-guided Argonaute protein directs RNA polymerase V to initiate DNA methylation. Nat. Plants7, 1461–1474 (2021). Initial establishment of DNA methylation is the least understood stage in RdDM. This article provides important evidence that an AGO protein binds to the target DNA and recruits Pol V. ArticleCASPubMedPubMed CentralGoogle Scholar
- McCue, A. D. et al. ARGONAUTE 6 bridges transposable element mRNA-derived siRNAs to the establishment of DNA methylation. EMBO J.33, 2737–2881 (2014). CASGoogle Scholar
- Melnyk, C. W., Molnar, A. & Baulcombe, D. C. Intercellular and systemic movement of RNA silencing signals. EMBO J.30, 3553–3563 (2011). ArticleCASPubMedPubMed CentralGoogle Scholar
- Schwach, F., Vaistij, F. E., Jones, L. & Baulcombe, D. C. An RNA-dependent RNA-polymerase prevents meristem invasion by potato virus X and is required for the activity but not the production of a systemic silencing signal. Plant. Physiol.138, 1842–1852 (2005). ArticleCASPubMedPubMed CentralGoogle Scholar
- Havelda, Z., Hornyik, C., Crescenzi, A. & Burgyan, J. In situ characterization of Cymbidium Ringspot Tombusvirus infection-induced posttranscriptional gene silencing in Nicotiana benthamiana. J. Virol.77, 6082–6086 (2003). ArticleCASPubMedPubMed CentralGoogle Scholar
- Kørner, C. J. et al. Crosstalk between PTGS and TGS pathways in natural antiviral immunity and disease recovery. Nat. Plants4, 157–164 (2018). ArticlePubMedCASGoogle Scholar
- Devers, E. A. et al. Movement and differential consumption of short interfering RNA duplexes underlie mobile RNA interference. Nat. Plants6, 789–799 (2020). This article provides clear evidence that mobile silencing is mediated by AGO-free sRNA duplexes that become bound by AGO proteins in the recipient cell. ArticleCASPubMedGoogle Scholar
- Sena, G., Jung, J. W. & Benfey, P. N. A broad competence to respond to SHORT ROOT revealed by tissue-specific ectopic expression. Development131, 2817–2826 (2004). ArticleCASPubMedGoogle Scholar
- Wafula, E. et al. MicroRNAs from the parasitic plant Cuscuta campestris target host messenger RNAs. Nature553, 82–85 (2018). ArticlePubMedCASGoogle Scholar
- Liu, L. & Chen, X. Intercellular and systemic trafficking of RNAs in plants. Nat. Plants4, 869–878 (2018). ArticlePubMedPubMed CentralGoogle Scholar
- Voinnet, O., Vain, P., Angell, S. & Baulcombe, D. C. Systemic spread of sequence-specific transgene RNA degradation is initiated by localised introduction of ectopic promoterless DNA. Cell95, 177–187 (1998). ArticleCASPubMedGoogle Scholar
- Vatén, A. et al. Callose biosynthesis regulates symplastic trafficking during root development. Dev. Cell21, 1144–1155 (2011). ArticlePubMedCASGoogle Scholar
- Liu, J., Zhang, L. & Yan, D. Plasmodesmata-involved battle against pathogens and potential strategies for strengthening hosts. Front. Plant. Sci.12, 644870 (2021). ArticlePubMedPubMed CentralGoogle Scholar
- Pyott, D. E. & Molnar, A. Going mobile: non-cell-autonomous small RNAs shape the genetic landscape of plants. Plant. Biotechnol. J.13, 306–318 (2015). ArticleCASPubMedGoogle Scholar
- Smith, N. A., Eamens, A. L. & Wang, M. B. Viral small interfering RNAs target host genes to mediate disease symptoms in plants. PLoS Pathog.7, 1–9 (2011). ArticleCASGoogle Scholar
- Shimura, H. et al. A viral satellite RNA induces yellow symptoms on tobacco by targeting a gene involved in chlorophyll biosynthesis using the RNA silencing machinery. PLoS Pathog.7, e1002021 (2011). ArticleCASPubMedPubMed CentralGoogle Scholar
- Adkar-Purushothama, C. R. et al. Small RNA derived from the virulence modulating region of the potato spindle tuber viroid silences callose synthase genes of tomato plants. Plant. Cell27, 2178–2194 (2015). ArticleCASPubMedPubMed CentralGoogle Scholar
- Cao, M. et al. Virus infection triggers widespread silencing of host genes by a distinct class of endogenous siRNAs in Arabidopsis. Proc. Natl Acad. Sci. USA111, 14613–14618 (2014). ArticleCASPubMedPubMed CentralGoogle Scholar
- Pitzalis, N. et al. Turnip mosaic virus in oilseed rape activates networks of sRNA-mediated interactions between viral and host genomes. Commun. Biol.3, 1–16 (2020). ArticleCASGoogle Scholar
- Fei, Y., Nyikó, T. & Molnar, A. Non-perfectly matching small RNAs can induce stable and heritable epigenetic modifications and can be used as molecular markers to trace the origin and fate of silencing RNAs. Nucleic Acids Res.49, 1900–1913 (2021). ArticleCASPubMedPubMed CentralGoogle Scholar
- Yang, Y. et al. Tomato yellow leaf curl virus intergenic siRNAs target a host long noncoding RNA to modulate disease symptoms. PLoS Pathog.15, 1–22 (2019). ArticleGoogle Scholar
- Döring, T. F. & Chittka, L. Visual ecology of aphids — a critical review on the role of colours in host finding. Arthropod Plant Interact.1, 3–16 (2007). ArticleGoogle Scholar
- Timmons, L. & Fire, A. Specific interference by ingested dsRNA. Nature395, 854 (1998). ArticleCASPubMedGoogle Scholar
- Banerjee, S. et al. RNA interference: a novel source of resistance to combat plant parasitic nematodes. Front. Plant. Sci.8, 1–8 (2017). ArticleGoogle Scholar
- Hudzik, C., Hou, Y., Ma, W. & Axtell, M. J. Exchange of small regulatory RNAs between plants and their pests. Plant. Physiol.182, 51–62 (2020). ArticleCASPubMedGoogle Scholar
- Nowara, D. et al. HIGS: host-induced gene silencing in the obligate biotrophic fungal pathogen Blumeria graminis. Plant. Cell22, 3130–3141 (2010). ArticleCASPubMedPubMed CentralGoogle Scholar
- Pliego, C. et al. Host-induced gene silencing in barley powdery mildew reveals a class of ribonuclease-like effectors. Mol. Plant Microbe Interact.26, 633–642 (2013). ArticleCASPubMedGoogle Scholar
- Zhang, H. et al. Functional characterization of calcineurin homologs PsCNA1/PsCNB1 in Puccinia striiformis f. sp. tritici using a host-induced RNAi system. PLoS ONE7, 1–8 (2012). CASGoogle Scholar
- Koch, A. et al. Host-induced gene silencing of cytochrome P450 lanosterol C14α-demethylase-encoding genes confers strong resistance to Fusarium species. Proc. Natl Acad. Sci. USA110, 19324–19329 (2013). ArticleCASPubMedPubMed CentralGoogle Scholar
- Panwar, V., McCallum, B. & Bakkeren, G. Host-induced gene silencing of wheat leaf rust fungus Puccinia triticina pathogenicity genes mediated by the Barley stripe mosaic virus. Plant Mol. Biol.81, 595–608 (2013). ArticleCASPubMedGoogle Scholar
- Ghag, S. B., Shekhawat, U. K. S. & Ganapathi, T. R. Host-induced post-transcriptional hairpin RNA-mediated gene silencing of vital fungal genes confers efficient resistance against Fusarium wilt in banana. Plant Biotechnol. J.12, 541–553 (2014). ArticleCASPubMedGoogle Scholar
- Andrade, C. M., Tinoco, M. L. P., Rieth, A. F., Maia, F. C. O. & Aragão, F. J. L. Host-induced gene silencing in the necrotrophic fungal pathogen Sclerotinia sclerotiorum. Plant Pathol.65, 626–632 (2016). ArticleCASGoogle Scholar
- Wang, M. et al. Bidirectional cross-kingdom RNAi and fungal uptake of external RNAs confer plant protection. Nat. Plants2, 16151 (2016). ArticleCASPubMedPubMed CentralGoogle Scholar
- Chen, W. et al. Host-induced silencing of Fusarium culmorum genes protects wheat from infection. J. Exp. Bot.67, 4979–4991 (2016). ArticleCASPubMedPubMed CentralGoogle Scholar
- Govindarajulu, M., Epstein, L., Wroblewski, T. & Michelmore, R. W. Host-induced gene silencing inhibits the biotrophic pathogen causing downy mildew of lettuce. Plant Biotechnol. J.13, 875–883 (2015). ArticleCASPubMedGoogle Scholar
- Jahan, S. N. et al. Plant-mediated gene silencing restricts growth of the potato late blight pathogen Phytophthora infestans. J. Exp. Bot.25, 265–288 (2015). Google Scholar
- Hou, Y. et al. A phytophthora effector suppresses trans-kingdom RNAi to promote disease susceptibility. Cell Host Microbe25, 153–165 (2019). ArticleCASPubMedGoogle Scholar
- Helber, N. et al. A versatile monosaccharide transporter that operates in the arbuscular mycorrhizal fungus Glomus sp is crucial for the symbiotic relationship with plants. Plant. Cell23, 3812–3823 (2011). ArticleCASPubMedPubMed CentralGoogle Scholar
- Singla-Rastogi, M. et al. Plant small RNA species direct gene silencing in pathogenic bacteria as well as disease protection. Preprint at bioRxivhttps://doi.org/10.1101/863902 (2019). ArticleGoogle Scholar
- He, B. et al. RNA-binding proteins contribute to small RNA loading in plant extracellular vesicles. Nat. Plants7, 342–352 (2021). ArticleCASPubMedPubMed CentralGoogle Scholar
- Cai, Q. et al. Plants send small RNAs in extracellular vesicles to silence virulence genes. Science360, 1126–1129 (2018). ArticleCASPubMedPubMed CentralGoogle Scholar
- Zhang, T. et al. Cotton plants export microRNAs to inhibit virulence gene expression in a fungal pathogen. Nat. Plants2, 1–6 (2016). ArticleGoogle Scholar
- Navarro, L., Jay, F., Nomura, K., He, S. Y. & Voinnet, O. Suppression of the microRNA pathway by bacterial effector proteins. Science321, 964–967 (2008). ArticleCASPubMedPubMed CentralGoogle Scholar
- Vetukuri, R. R., Whisson, S. C. & Grenville-Briggs, L. J. Phytophthora infestans effector Pi14054 is a novel candidate suppressor of host silencing mechanisms. Eur. J. Plant. Pathol.149, 771–777 (2017). ArticleCASGoogle Scholar
- Qiao, Y. et al. Oomycete pathogens encode RNA silencing suppressors. Nat. Genet.45, 1–6 (2013). ArticleCASGoogle Scholar
- Qiao, Y., Shi, J., Zhai, Y., Hou, Y. & Ma, W. Phytophthora effector targets a novel component of small RNA pathway in plants to promote infection. Proc. Natl Acad. Sci. USA15, 5850–5855 (2015). ArticleCASGoogle Scholar
- Xiong, Q. et al. Phytophthora suppressor of RNA silencing 2 is a conserved RxLR effector that promotes infection in soybean and Arabidopsis thaliana. Mol. Plant-Microbe Interact.27, 1379–1389 (2014). ArticlePubMedCASGoogle Scholar
- Yin, C. et al. A novel fungal effector from Puccinia graminis suppressing RNA silencing and plant defense responses. N. Phytol.222, 1561–1572 (2019). ArticleCASGoogle Scholar
- Weiberg, A. et al. Fungal small RNAs suppress plant immunity by hijacking host RNA interference pathways. Science342, 118–123 (2013). ArticleCASPubMedPubMed CentralGoogle Scholar
- Wang, B. et al. Puccinia striiformis f. sp. tritici microRNA-like RNA 1 (Pst-milR1), an important pathogenicity factor of Pst, impairs wheat resistance to Pst by suppressing the wheat pathogenesis-related 2 gene. N. Phytol.215, 338–350 (2017). ArticleCASGoogle Scholar
- Jian, J. & Liang, X. One small RNA of Fusarium graminearum targets and silences CEBiP gene in common wheat. Microorganisms7, 1–12 (2019). ArticleCASGoogle Scholar
- Dunker, F. et al. Oomycete small RNAs bind to the plant RNA-induced silencing complex for virulence. Elife9, e56096 (2020). ArticlePubMedPubMed CentralGoogle Scholar
- Hu, X., Persson Hodén, K., Liao, Z., Åsman, A. & Dixelius, C. Phytophthora infestans Ago1-associated miRNA promotes potato late blight disease. N. Phytol.233, 443–457 (2022). ArticleCASGoogle Scholar
- Baldrich, P. et al. Plant extracellular vesicles contain diverse small RNA species and are enriched in 10- to 17-nucleotide “tiny” RNAs. Plant Cell31, 315–324 (2019). ArticleCASPubMedPubMed CentralGoogle Scholar
- Ren, B., Wang, X., Duan, J. & Ma, J. Rhizobial tRNA-derived small RNAs are signal molecules regulating plant nodulation. Science365, 919–922 (2019). ArticleCASPubMedGoogle Scholar
- Wong-Bajracharya, J. et al. The ectomycorrhizal fungus Pisolithus microcarpus encodes a microRNA involved in cross-kingdom gene silencing during symbiosis. Proc. Natl Acad. Sci. USA119, e2103527119 (2022). ArticleCASPubMedPubMed CentralGoogle Scholar
- Cai, Q. et al. Message in a bubble: shuttling small RNAs and proteins between cells and interacting organisms using extracellular vesicles. Annu. Rev. Plant Biol.72, 497–524 (2021). ArticleCASPubMedPubMed CentralGoogle Scholar
- Rutter, B. D. & Innes, R. W. Growing pains: addressing the pitfalls of plant extracellular vesicle research. N. Phytol.228, 1505–1510 (2020). ArticleGoogle Scholar
- Karimi, H. Z. et al. Arabidopsis apoplastic fluid contains sRNA- and circular RNA-protein complexes that are located outside extracellular vesicles. Plant. Cellhttps://doi.org/10.1093/plcell/koac043 (2022). This article provides controversial findings leading to the conclusion that transkingdom RNA may be transported outside extracellular vesicles. This extracellular RNA is enriched inN6-methyladenine and associated with GRP7 and AGO2. ArticleGoogle Scholar
- Roth, R. et al. Arbuscular cell invasion coincides with extracellular vesicles and membrane tubules. Nat. Plants5, 204–211 (2019). ArticleCASPubMedGoogle Scholar
- Ludwig, N. et al. A cell surface-exposed protein complex with an essential virulence function in Ustilago maydis. Nat. Microbiol.6, 722–730 (2021). ArticleCASPubMedPubMed CentralGoogle Scholar
- Laurie, J. D., Linning, R. & Bakkeren, G. Hallmarks of RNA silencing are found in the smut fungus Ustilago hordei but not in its close relative Ustilago maydis. Curr. Genet.53, 49–58 (2008). ArticleCASPubMedGoogle Scholar
- Taliansky, M. et al. RNA-based technologies for engineering plant virus resistance. Plants10, 1–19 (2021). ArticleCASGoogle Scholar
- Qiao, L. et al. Spray-induced gene silencing for disease control is dependent on the efficiency of pathogen RNA uptake. Plant Biotechnol. J.19, 1756–1768 (2021). ArticleCASPubMedPubMed CentralGoogle Scholar
- Johnson, N. R., de Pamphilis, C. W. & Axtell, M. J. Compensatory sequence variation between trans-species small RNAs and their target sites. eLife8, e49750 (2019). ArticlePubMedPubMed CentralGoogle Scholar
- Rose, L. E., Overdijk, E. J. R. & van Damme, M. Small RNA molecules and their role in plant disease. Eur. J. Plant Pathol.153, 115–128 (2019). ArticleCASGoogle Scholar
- Dora, S., Terrett, O. M. & Sánchez-Rodríguez, C. Plant–microbe interactions in the apoplast: communication at the plant cell wall. Plant Cellhttps://doi.org/10.1093/plcell/koac040 (2022). ArticlePubMedPubMed CentralGoogle Scholar
- Serrano, M., Coluccia, F., Torres, M., L’Haridon, F. & Métraux, J. P. The cuticle and plant defense to pathogens. Front. Plant Sci.5, 1–8 (2014). ArticleGoogle Scholar
- Bacete, L., Mélida, H., Miedes, E. & Molina, A. Plant cell wall-mediated immunity: cell wall changes trigger disease resistance responses. Plant J.93, 614–636 (2018). ArticleCASPubMedGoogle Scholar
- Osbourn, A. E. Preformed antimicrobial compounds and plant defense against fungal attack. Plant Cell8, 1821–1831 (1996). ArticleCASPubMedPubMed CentralGoogle Scholar
- Kourelis, J. & Van Der Hoorn, R. A. L. Defended to the nines: 25 years of resistance gene cloning identifies nine mechanisms for R protein function. Plant Cell30, 285–299 (2018). ArticleCASPubMedPubMed CentralGoogle Scholar
- Bentham, A. R. et al. A molecular roadmap to the plant immune system. J. Biol. Chem.295, 14916–14935 (2020). ArticleCASPubMedPubMed CentralGoogle Scholar
- Yuan, M. et al. Pattern-recognition receptors are required for NLR-mediated plant immunity. Nature592, 105–109 (2021). CASPubMedPubMed CentralGoogle Scholar
- Ngou, B. P. M., Ahn, H. K., Ding, P. & Jones, J. D. G. Mutual potentiation of plant immunity by cell-surface and intracellular receptors. Nature592, 110–115 (2021). ArticleCASPubMedGoogle Scholar
- Tian, H. et al. Activation of TIR signalling boosts pattern-triggered immunity. Nature598, 500–503 (2021). ArticleCASPubMedGoogle Scholar
- Hou, S., Liu, Z., Shen, H. & Wu, D. Damage-associated molecular pattern-triggered immunity in plants. Front. Plant Sci.10, 646 (2019). ArticlePubMedPubMed CentralGoogle Scholar
- Sacristán, S. & García-Arenal, F. The evolution of virulence and pathogenicity in plant pathogen populations. Mol. Plant Pathol.9, 369–384 (2008). ArticlePubMedPubMed CentralGoogle Scholar
- Zhou, T. et al. Domains of the cucumber mosaic virus 2b silencing suppressor protein affecting inhibition of salicylic acid-induced resistance and priming of salicylic acid accumulation during infection. J. Gen. Virol.95, 1408–1413 (2014). ArticleCASPubMedPubMed CentralGoogle Scholar
- Ji, L. H. & Ding, S. W. The suppressor of transgene RNA silencing encoded by Cucumber mosaic virus interferes with salicylic acid-mediated virus resistance. Mol. Plant Microbe Interact.14, 715–724 (2001). ArticleCASPubMedGoogle Scholar
- Love, A. J. et al. Cauliflower mosaic virus protein P6 inhibits signaling responses to salicylic acid and regulates innate immunity. PLoS ONE7, e47535 (2012). ArticleCASPubMedPubMed CentralGoogle Scholar
- Glick, E. et al. Interaction with host SGS3 is required for suppression of RNA silencing by tomato yellow leaf curl virus V2 protein. Proc. Natl Acad. Sci. USA105, 157 (2008). ArticleCASPubMedGoogle Scholar
- Wang, Y. et al. A calmodulin-binding transcription factor links calcium signaling to antiviral RNAi defense in plants. Cell Host Microbe29, 1393–1406 (2021). This article provides a clear illustration of how VSRs mediate connections between RNA silencing and other defence systems. ArticleCASPubMedGoogle Scholar
- Shan, L. et al. Bacterial effectors target the common signaling partner BAK1 to disrupt multiple MAMP receptor-signaling complexes and impede plant immunity. Cell Host Microbe4, 17–27 (2008). ArticleCASPubMedPubMed CentralGoogle Scholar
- He, J. et al. Structural analysis of Phytophthora suppressor of RNA silencing 2 (PSR2) reveals a conserved modular fold contributing to virulence. Proc. Natl Acad. Sci. USA116, 8054–8059 (2019). This article provides structural information about a suppressor of silencing from the late blightPhytophthoraoomycete. ArticleCASPubMedPubMed CentralGoogle Scholar
- Wu, D. et al. Viral effector protein manipulates host hormone signaling to attract insect vectors. Cell Res.27, 402–415 (2017). ArticleCASPubMedPubMed CentralGoogle Scholar
- Ziebell, H. et al. Cucumber mosaic virus and its 2b RNA silencing suppressor modify plant-aphid interactions in tobacco. Sci. Rep.1, 1–7 (2011). ArticleCASGoogle Scholar
- Malcuit, I. et al. The 25-kDa movement protein of PVX elicits Nb-mediated hypersensitive cell death in potato. Mol. Plant Microbe Interact.12, 536–543 (1999). ArticleCASGoogle Scholar
- Voinnet, O., Lederer, C. & Baulcombe, D. C. A viral movement protein prevents spread of the gene silencing signal in Nicotiana benthamiana. Cell103, 157–167 (2000). ArticleCASPubMedGoogle Scholar
- de Ronde, D. et al. Analysis of Tomato spotted wilt virus NSs protein indicates the importance of the N-terminal domain for avirulence and RNA silencing suppression. Mol. Plant Pathol.15, 185–195 (2014). ArticlePubMedCASGoogle Scholar
- Ren, T., Qu, F. & Morris, T. J. HRT gene function requires interaction between a NAC protein and viral capsid protein to confer resistance to turnip crinkle virus. Plant Cell12, 1917–1926 (2000). ArticleCASPubMedPubMed CentralGoogle Scholar
- Zhai, J. et al. MicroRNAs as master regulators of the plant NB-LRR defense gene family via the production of phased, trans-acting siRNAs. Genes Dev.25, 2540–2553 (2011). ArticleCASPubMedPubMed CentralGoogle Scholar
- Shivaprasad, P. V. et al. A microRNA superfamily regulates nucleotide binding site-leucine-rich repeats and other mRNAs. Plant Cell24, 859–874 (2012). ArticleCASPubMedPubMed CentralGoogle Scholar
- Liu, J. et al. The miR9863 family regulates distinct Mla alleles in barley to attenuate NLR receptor-triggered disease resistance and cell-death signaling. PLoS Genet.10, e1004755 (2014). ArticlePubMedPubMed CentralCASGoogle Scholar
- Li, F. et al. MicroRNA regulation of plant innate immune receptors. Proc. Natl Acad. Sci. USA109, 1790–1795 (2011). ArticleGoogle Scholar
- Liu, Y., Teng, C., Xia, R. & Meyers, B. C. PhasiRNAs in plants: their biogenesis, genic sources, and roles in stress responses, development, and reproduction. Plant Cell32, 3059–3080 (2020). ArticleCASPubMedPubMed CentralGoogle Scholar
- Canto-Pastor, A. et al. Enhanced resistance to bacterial and oomycete pathogens by short tandem target mimic RNAs in tomato. Proc. Natl Acad. Sci. USA116, 2755–2760 (2019). ArticleCASPubMedPubMed CentralGoogle Scholar
- Deng, Y. et al. A role for small RNA in regulating innate immunity during plant growth. PLoS Pathog.14, e1006756 (2018). ArticlePubMedPubMed CentralCASGoogle Scholar
- Cui, C. et al. A Brassica miRNA regulates plant growth and immunity through distinct modes of action. Mol. Plant13, 231–245 (2020). ArticleCASPubMedGoogle Scholar
- Vaucheret, H., Mallory, A. C. & Bartel, D. P. AGO1 homeostasis entails coexpression of MIR168 and AGO1 and preferential stabilization of miR168 by AGO1. Mol. Cell22, 129–136 (2006). ArticleCASPubMedPubMed CentralGoogle Scholar
- Xie, Z., Kasschau, K. D. & Carrington, J. C. Negative feedback regulation of Dicer-Like1 in Arabidopsis by microRNA-guided mRNA degradation. Curr. Biol.13, 784–789 (2003). ArticleCASPubMedGoogle Scholar
- Harvey, J. J. W. et al. An antiviral defense role of AGO2 in plants. PLoS ONE6, e14639 (2011). ArticleCASPubMedPubMed CentralGoogle Scholar
- Jiang, N., Meng, J., Cui, J., Sun, G. & Luan, Y. Function identification of miR482b, a negative regulator during tomato resistance to Phytophthora infestans. Hortic. Res.5, 9 (2018). Canto-Pastor et al. (2019) and Jiang et al. (2018) illustrate how miRNA-mediated targeting of NLRs leads to suppression of basal resistance in uninfected plants, and the potential of pathogen-encoded suppressors of silencing to enhance immunity in infected plants. ArticleCASPubMedPubMed CentralGoogle Scholar
- Boccara, M. The Arabidopsis miR472-RDR6 silencing pathway modulates PAMP- and effector-triggered immunity through the post-transcriptional control of disease resistance genes. PLoS Pathog. 11, e1004814 (2014). ArticleCASGoogle Scholar
- Nakahara, K. S. et al. Tobacco calmodulin-like protein provides secondary defense by binding to and directing degradation of virus RNA silencing suppressors. Proc. Natl Acad. Sci. USA109, 10113–10118 (2012). ArticleCASPubMedPubMed CentralGoogle Scholar
- Navarro, L. et al. A plant miRNA contributes to antibacterial resistance by repressing auxin signaling. Science312, 436 (2006). ArticleCASPubMedGoogle Scholar
- Katiyar-Agarwal, S. et al. A pathogen-inducible endogenous siRNA in plant immunity. Proc. Natl Acad. Sci. USA103, 18002–18007 (2006). ArticleCASPubMedPubMed CentralGoogle Scholar
- Campo, S. et al. Identification of a novel microRNA (miRNA) from rice that targets an alternatively spliced transcript of the Nramp6 (natural resistance-associated macrophage protein 6) gene involved in pathogen resistance. N. Phytol.199, 212–227 (2013). ArticleCASGoogle Scholar
- Zhang, X. et al. Arabidopsis argonaute 2 regulates innate immunity via miRNA393*-mediated silencing of a Golgi-localized SNARE gene, MEMB12. Mol. Cell42, 356–366 (2011). ArticleCASPubMedPubMed CentralGoogle Scholar
- Li, Y. et al. Identification of microRNAs involved in pathogen-associated molecular pattern-triggered plant innate immunity. Plant Physiol.152, 2222–2231 (2010). ArticleCASPubMedPubMed CentralGoogle Scholar
- Yang, Z. et al. Jasmonate signaling enhances RNA silencing and antiviral defense in rice. Cell Host Microbe28, 89–103 (2020). ArticleCASPubMedGoogle Scholar
- Wu, J. et al. Viral-inducible Argonaute18 confers broad-spectrum virus resistance in rice by sequestering a host microRNA. eLife4, e05733 (2015). This article provides a nice illustration of a complex network of defence systems in plants: RNA silencing is an important effector mechanism and integrator of the different systems. ArticlePubMed CentralCASGoogle Scholar
- Dowen, R. H. et al. Widespread dynamic DNA methylation in response to biotic stress. Proc. Natl Acad. Sci. USA109, E2183–E2191 (2012). ArticleCASPubMedPubMed CentralGoogle Scholar
- Yu, A. et al. Dynamics and biological relevance of DNA demethylation in Arabidopsis antibacterial defense. Proc. Natl Acad. Sci. USA110, 2389–2394 (2013). ArticleCASPubMedPubMed CentralGoogle Scholar
- Akimoto, K. et al. Epigenetic inheritance in rice plants. Ann. Bot.100, 205–217 (2007). ArticleCASPubMedPubMed CentralGoogle Scholar
- Corrêa, R. L. et al. Viral fitness determines the magnitude of transcriptomic and epigenomic reprograming of defense responses in plants. Mol. Biol. Evol.37, 1866–1881 (2020). ArticlePubMedCASGoogle Scholar
- Jaskiewicz, M., Conrath, U. & Peterhälnsel, C. Chromatin modification acts as a memory for systemic acquired resistance in the plant stress response. EMBO Rep.12, 50–55 (2011). ArticleCASPubMedGoogle Scholar
- Schillheim, B. et al. Sulforaphane modifies histone H3, unpacks chromatin, and primes defense. Plant Physiol.176, 2395–2405 (2018). ArticleCASPubMedGoogle Scholar
- Jin, H. et al. Salicylic acid-induced transcriptional reprogramming by the HAC-NPR1-TGA histone acetyltransferase complex in Arabidopsis. Nucleic Acids Res.46, 11712–11725 (2018). CASPubMedPubMed CentralGoogle Scholar
- Halter, T. et al. The Arabidopsis active demethylase ros1 cis-regulates defense genes by erasing DNA methylation at promoter-regulatory regions. eLife10, e62994 (2021). This article provides new findings showing the importance of active DNA demethylation in PTI and basal resistance. ArticleCASPubMedPubMed CentralGoogle Scholar
- López Sánchez, A., Stassen, J. H. M., Furci, L., Smith, L. M. & Ton, J. The role of DNA (de)methylation in immune responsiveness of Arabidopsis. Plant J.88, 361–374 (2016). ArticlePubMedPubMed CentralCASGoogle Scholar
- Geng, S. et al. DNA methylation dynamics during the interaction of wheat progenitor Aegilops tauschii with the obligate biotrophic fungus Blumeria graminis f. sp. tritici. N. Phytol.221, 1023–1035 (2019). ArticleCASGoogle Scholar
- Satgé, C. et al. Reprogramming of DNA methylation is critical for nodule development in Medicago truncatula. Nat. Plants2, 1–10 (2016). ArticleCASGoogle Scholar
- Conrath, U., Beckers, G. J. M., Langenbach, C. J. G. & Jaskiewicz, M. R. Priming for enhanced defense. Annu. Rev. Phytopathol.53, 97–119 (2015). ArticleCASPubMedGoogle Scholar
- Luna, E., Bruce, T. J. A., Roberts, M. R., Flors, V. & Ton, J. Next-generation systemic acquired resistance. Plant Physiol.158, 844–853 (2012). ArticleCASPubMedGoogle Scholar
- Slaughter, A. et al. Descendants of primed Arabidopsis plants exhibit resistance to biotic stress. Plant Physiol.158, 835–843 (2012). ArticleCASPubMedGoogle Scholar
- Ando, S. et al. Priming for enhanced ARGONAUTE2 activation accompanies induced resistance to cucumber mosaic virus in Arabidopsis thaliana. Mol. Plant Pathol.22, 19–30 (2021). ArticleCASPubMedGoogle Scholar
- Beckers, G. J. M. et al. Mitogen-activated protein kinases 3 and 6 are required for full priming of stress responses in Arabidopsis thaliana. Plant Cell21, 944–953 (2009). ArticleCASPubMedPubMed CentralGoogle Scholar
- Pick, T., Jaskiewicz, M., Peterhänsel, C. & Conrath, U. Heat shock factor HsfB1 primes gene transcription and systemic acquired resistance in Arabidopsis. Plant Physiol.159, 52–55 (2012). ArticleCASPubMedPubMed CentralGoogle Scholar
- Baum, S. et al. Isolation of open chromatin identifies regulators of systemic acquired Resistance. Plant Physiol.181, 817–833 (2019). ArticleCASPubMedPubMed CentralGoogle Scholar
- Furci, L. et al. Identification and characterisation of hypomethylated DNA loci controlling quantitative resistance in Arabidopsis. eLife8, e40655 (2019). Not directly related to RNA silencing, this study illustrates priming of immunity by prior exposure to a pathogen. ArticlePubMedPubMed CentralGoogle Scholar
- Voinnet, O. RNA silencing as a plant immune system against viruses. Trends Genet.17, 449–459 (2001). ArticleCASPubMedGoogle Scholar
- Tamborski, J. & Krasileva, K. V. Evolution of plant NLRs: from natural history to precise modifications. Annu. Rev. Plant Biol.71, 355–378 (2020). ArticleCASPubMedGoogle Scholar
- Zhao, M., Meyers, B. C., Cai, C., Xu, W. & Ma, J. Evolutionary patterns and coevolutionary consequences of MIRNA genes and microRNA targets triggered by multiple mechanisms of genomic duplications in soybean. Plant Cell27, 546–562 (2015). ArticleCASPubMedPubMed CentralGoogle Scholar
- González, V. M. V. M., Müller, S., Baulcombe, D. C. & Puigdomènech, P. Evolution of NBS-LRR gene copies among dicot plants and its regulation by members of the miR482/2118 superfamily of miRNAs. Mol. Plant.8, 329–331 (2015). ArticlePubMedCASGoogle Scholar
- Deng, Y. et al. Epigenetic regulation of antagonistic receptors confers rice blast resistance with yield balance. Science355, 962–965 (2017). ArticleCASPubMedGoogle Scholar
- Karasov, T. L., Chae, E., Herman, J. J. & Bergelson, J. Mechanisms to mitigate the trade-off between growth and defense. Plant Cell29, 666–680 (2017). ArticleCASPubMedPubMed CentralGoogle Scholar
- Kover, P. X. & Schaal, B. A. Genetic variation for disease resistance and tolerance among Arabidopsis thaliana accessions. Proc. Natl Acad. Sci. USA99, 11270–11274 (2002). ArticleCASPubMedPubMed CentralGoogle Scholar
- Poland, J. A., Balint-Kurti, P. J., Wisser, R. J., Pratt, R. C. & Nelson, R. J. Shades of gray: the world of quantitative disease resistance. Trends Plant Sci.14, 21–29 (2009). ArticleCASPubMedGoogle Scholar
- Mi, S. J. et al. Sorting of small RNAs into Arabidopsis argonaute complexes is directed by the 5′ terminal nucleotide. Cell133, 116–127 (2008). ArticleCASPubMedPubMed CentralGoogle Scholar
- Borsani, O., Zhu, J. K., Verslues, P. E., Sunkar, R. & Zhu, J. K. Endogenous siRNAs derived from a pair of natural cis-antisense transcripts regulate salt tolerance in Arabidopsis. Cell123, 1279–1291 (2005). ArticleCASPubMedPubMed CentralGoogle Scholar
- Allen, E., Xie, Z., Gustafson, A. M. & Carrington, J. C. microRNA-directed phasing during trans-acting siRNA biogenesis in plants. Cell121, 207–221 (2005). ArticleCASPubMedGoogle Scholar
- Borges, F. et al. Transposon-derived small RNAs triggered by miR845 mediate genome dosage response in Arabidopsis. Nat. Genet.50, 186–192 (2018). ArticleCASPubMedPubMed CentralGoogle Scholar
- Bond, D. M. & Baulcombe, D. C. Small RNAs and heritable epigenetic variation in plants. Trends Cell Biol.24, 100–107 (2014). ArticleCASPubMedGoogle Scholar
- Yang, X. et al. Suppression of methylation-mediated transcriptional gene silencing by βC1-SAHH protein interaction during Geminivirus-Betasatellite infection. PLoS Pathog.7, e1002329 (2011). ArticleCASPubMedPubMed CentralGoogle Scholar
- Hao, L., Wang, H., Sunter, G. & Bisaro, D. M. Geminivirus AL2 and L2 proteins interact with and inactivate SNF1 kinase. Plant Cell15, 1034–1048 (2003). ArticleCASPubMedPubMed CentralGoogle Scholar
- Bayne, E. H., Rakitina, D. V., Morozov, S. Y. & Baulcombe, D. C. Cell-to-cell movement of potato Potexvirus X is dependent on suppression of RNA silencing. Plant. J.44, 471–482 (2005). ArticleCASPubMedGoogle Scholar
- Chiu, M. H., Chen, I. H., Baulcombe, D. C. & Tsai, C. H. The silencing suppressor P25 of potato virus X interacts with Argonaute1 and mediates its degradation through the proteasome pathway. Mol. Plant. Pathol.11, 641–649 (2010). CASPubMedPubMed CentralGoogle Scholar
Acknowledgements
The authors’ research has been supported by European Research Council Advanced Investigator grant ERC-2013-AdG 340642 (Transgressive Inheritance in Plant Breeding and Evolution (TRIBE)), the Royal Society (RP170001), the Balzan Foundation, the Biological Sciences and Biotechnology Research Council (BB/R018529/1) and the Broodbank Fund. S.L.-G. is a Senior Broodbank Research Fellow. D.C.B. is the Royal Society Edward Penley Abraham Research Professor.